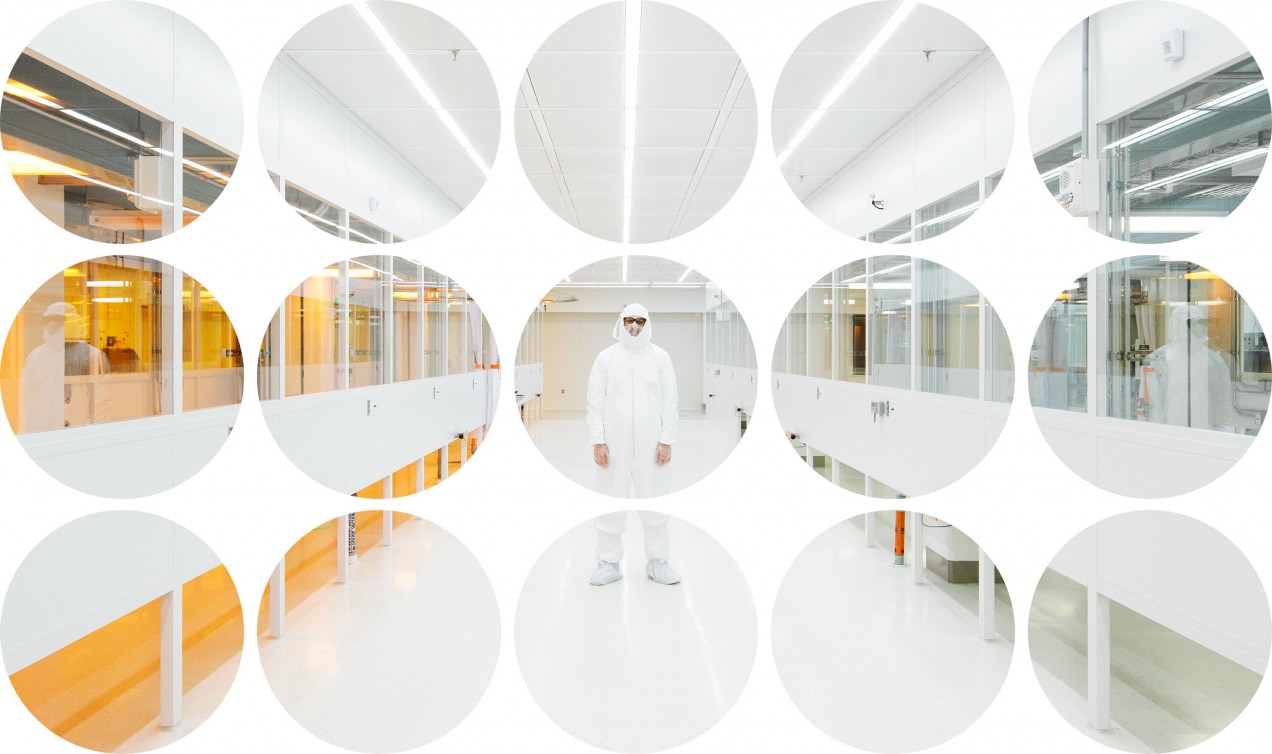
Two stories underground in MIT’s newest building, two biology postdocs and a graduate student are gathered around a computer monitor that shows a gray background flecked with little squiggles and a few dark blobs. The squiggles are diaphanous strands of proteins. The blobs are minuscule aggregations of ice that formed when the scientists flash-froze the proteins to unlock secrets that their strands have been hiding for hundreds of millions of years.
The protein strands are less than 50 nanometers in height and width. They appear on the screen because they’re being probed by a cryo-EM—a cryogenic electron microscope—in the next room.
Getting to this point requires some luck, because the proteins can get obscured or lost entirely in the freezing process. Researchers can spend days in this basement room with hardly any usable images coming up on the screen.
Now that the scientists have been fortunate enough to see so many squiggles, their next trick is to spot the few that are worth studying more closely. In living cells, these protein strands form a Y shape. That Y is a crucial component of what’s called the nuclear pore, which determines, in ways we still don’t understand, what can travel in and out of a cell’s nucleus. However, the proteins rarely have that Y shape on the screen. Right before they’re encased in the layer of ice that lets them be scanned by the cryo-EM, the arms and stalk of each Y tend to go noodly. One arm might be bent backwards while the other one dangles to the side. Multiple Ys or bits of them often clump together.
So today grad student Sarah Nordeen is patiently searching for one that’s in the form of a definitively clean and clear Y.
“I see one—a pretty one,” she says. Anthony Schuller, a postdoc structural biologist who is operating the computer, zooms a little closer to the squiggle she indicates. With a few mouse clicks, he tells the cryo-EM to capture close-up images that Nordeen can analyze later. If she can get enough of these nicely preserved Ys, eventually she can combine them to produce a 3-D rendering of this structure—which will help her and other scientists better understand how it serves as a gatekeeper inside cells.
This is life in MIT.nano, a facility that opened last fall in the shadow of the Great Dome. With two cryo-EM machines and other equipment that will be installed in the coming years, scientists in a widening range of disciplines will model, build, and repair things at atomic and molecular scales.
Some scientists will use MIT.nano to develop sturdier qubits for quantum computers. Others can tinker with the molecular makeup of anodes and cathodes so they don’t fray, lengthening the life of batteries. Still other groups hope to create materials optimized for specific functions by tailoring their molecular structures to do such things as conduct electricity more effectively, create more lustrous colors on computer displays, or dispense targeted drugs in the bloodstream. MIT.nano will even have a nanoscale arts program. Artists can use materials generated by MIT.nano researchers, or take advantage of tools in the building to exercise pinpoint control over the ways that objects shimmer, feel, or smell.
All these applications are possible because of better and better techniques for imaging materials at the atomic level. “We make new ways of seeing, and then we see new ways of making,” President L. Rafael Reif said at the MIT.nano launch ceremony in October.
To drive home the interdisciplinary aspect of it all, no faculty will have offices in the $400 million MIT.nano building; only a few dozen staffers who will oversee the equipment will be based there. The microscopes, clean rooms, and fabrication facilities are meant to be used by people from departments all over campus. The dedicated space means new equipment won’t need to be wedged into already-packed labs, and multiple versions of critical machines can be run simultaneously without danger of cross-contamination, expanding the capacity for research. It also means researchers will have access to cutting-edge equipment that would be too expensive to run and maintain in their own labs—and it won’t sit idle when they’re not using it.
For months after it opened, most of MIT.nano was empty. The facility—Building 12, right off the Infinite Corridor at the heart of campus—was nonetheless impressive, a sleek glass-and-steel structure that looks out onto a walkway with bamboo and birch trees. (It’s called the Improbability Walk in honor of the late Institute Professor and nano pioneer Mildred Dresselhaus, who once described her own career as improbable, given her humble beginnings.) But if you looked in, you could see clean rooms and lab space waiting to be used. It takes time to transfer some of MIT’s most advanced tools for observing and building nanoscale things from Building 39, home of the Microsystems Technology Laboratories, and to identify—and raise the funds for—newer equipment worth investing in.
Aside from the bustling undergraduate chemistry labs on the top floor of the building, the early action at MIT.nano was in the basement. To allow cryo-EMs and similar instruments to work, the basement has special rooms that are shielded from electromagnetic radiation—you can’t get a cell phone signal—and outfitted with platforms that cancel out vibrations from the building and the world outside. A cryo-EM machine costs about $5 million; the room that houses it is an additional three or four million. Having two on campus is a welcome change. Before MIT.nano opened, MIT researchers had to borrow time on older models of cryo-EMs at other institutions.
Scientists have been peeking at things at this scale for a very long time. X-ray crystallography, for example, arose a century ago. That’s what made it possible to determine the structure of DNA in 1953. Nuclear magnetic resonance spectroscopy, which can be used to ascertain a compound’s atomic structure, and electron microscopes, which fire a beam of electrons at an object and measure how they scatter, were developed in the 1950s. In the 1980s came scanning-tunneling microscopes, which can image individual atoms in a conductive material. STMs work by hovering an ultra-sharp tip just above the sample and measuring the current of the electrons that “tunnel” from the tip to the material. Then came the atomic force microscope, which has even higher resolution. It can push and prod atoms and molecules, as well as witness activity in non-conducting samples, including living cells.
Impressive as those methods all are, they have been blind to a vast amount of biological material, much of which doesn’t lend itself well to being crystallized or bombarded with high amounts of energy. Cryo-EMs, based on breakthroughs that won the Nobel Prize in chemistry in 2017, have proved particularly useful for examining in great detail the gooey stuff inside cells.
Although cryo-EM technology began to emerge in the 1980s and 1990s, it’s gotten significantly better in the past few years. Improvements in camera technologies have allowed researchers to improve the resolution five- or 10-fold: cryo-EMs can now resolve images smaller than 3 angstroms. (An angstrom, a tenth of nanometer, is the diameter of a hydrogen atom.) And the picture should get much sharper before long. The theoretical limits of the technique haven’t yet been hit, and technologies now in development could limit the damage the electron beams in these machines inflict on the samples being examined, says Edward Brignole, who oversees the cryo-EMs in MIT.nano.
The Y-shaped protein being analyzed in MIT.nano first came into view about a decade ago, thanks to crystallography and other techniques. That was a crucial start for figuring out what it does in the nuclear pore, since a protein’s function is dictated by the structures that are naturally formed by its chains of amino acids. But only with the tools available now can scientists see both the Y itself and the connections it makes to other subunits of the nuclear pore.
For a sense of just how small the scale is here, consider that the Y structure is made up of only about 100,000 atoms, according to Thomas Schwartz, the MIT structural biologist whose lab includes Nordeen and Schuller. If we better understood how it fits with the other pieces, we could learn just how the pore allows, say, messenger RNA to get out of the nucleus and proteins to get in. We could also find out why its gatekeeping methods aren’t foolproof. How do some viruses get into the nucleus, where they replicate? Is there a way to stop that?
Other biologists taking turns on the cryo-EMs have different questions. Postdoc Xue Fei is using it to study proteins that bacteria use to dispose of waste. Kacper Rogala, a postdoc at the Whitehead Institute for Biomedical Research, which is affiliated with MIT, is examining individual pieces of the mTOR pathway. That is a signaling mechanism that regulates the metabolism of cells. It has been linked to cancer and longevity, and maybe it’ll be possible to develop drugs that target very specific interactions in this pathway rather than the entire thing.
“We are just at the very early, early, early days of exploiting the opportunities of the nanoscale,” says Vladimir Bulović, the engineering professor who is director of MIT.nano. We’re sitting in his homey office, with high cabinets, plants, and a wooden coffee table, in Building 13, down the hall from a newly opened corridor that connects to an upper floor of MIT.nano. He’s explaining why the equipment that will eventually fill the building will lay the groundwork for applications we can’t necessarily imagine yet.
Bulović has done pioneering work on materials such as quantum dots, nano-size semiconductor particles that are useful in high-definition TVs, solar cells, and biological research. They exploit quantum mechanics in ways that couldn’t be directly observed before the scanning tunneling microscope was invented in the 1980s. But as Bulović points out, it took a long time for that particular breakthrough in visualization to be used in products. At first, “we spent our time just being happy to see atoms,” he says. Mastery over the atoms came slowly. In 1993, IBM scientists managed to corral atoms into configurations that manipulated the behavior of electrons. But it wasn’t until the 2000s, Bulović says, that scientists became adept at harnessing the STM’s powers of observation for the design of specific materials.
Now he expects to see a similar process unfold in MIT.nano, where machines that have generally been used for highly specialized experiments will become “ready-made tool sets” for wider use.
One of the professors going from seeing to making is Farnaz Niroui, SM ’13, PhD ’17, who just joined the engineering faculty. She is able to precisely control how electrons interact with each other inside materials designed at the nanoscale. That lays the groundwork for devices that are much more energy efficient than what we have today. Meanwhile, materials science and engineering professor Frances Ross and her colleagues are documenting exactly what happens when certain kinds of conductive molecules self-organize into nanowires. If such insights make it possible to grow wires inside new materials, who knows what electronic devices will be made out of them?
To give another example, Bulović reaches for a piece of plastic with black rectangles on it. It’s a prototype of a flexible yet ultra-efficient type of solar cell that absorbs more wavelengths of light than today’s photovoltaic devices do. Making it has required scientists to tinker with the molecular properties of minerals known as perovskites, which can harvest solar energy. Bulović says people walking by MIT.nano in the coming years might peer in and see scientists smearing perovskite-rich pastes onto plastic sheets as they try to perfect the technology.
Bulović mentions that possibility to highlight the clean-energy research being pursued through MIT’s interdisciplinary programs such as GridEdge Solar, which aims to scale up the production of lightweight, flexible solar cells. But he also brings it up to note the transparent nature of MIT.nano. Much of what will happen inside, other than the biological research, will be a continuation of work that has been active for years in the Microsystems Technology Labs—a department that Bulović, Reif, and provost Martin Schmidt each once directed. But here, Bulović says, things will be more open.
Bulović recalls being visited several years ago by a director of movies who said, “You know, Vladimir, you guys here at MIT, you’re kind of like a cauldron of mystery. Stuff just kind of pops out of the cauldron, but we don’t really know what the soup is. Show us the soup!” It’s in that spirit, Bulović says, that there are windows everywhere.
“We want to make sure you can peek in,” he says. “You won’t understand exactly what’s happening, but you’ll see activity. You’ll see people dedicated to their craft, and you’ll marvel at how they do, X, Y, or Z.”
Just as important, he says, is for the people inside the clean rooms and other labs to see out. “We ensure that you can look outside MIT.nano and see the shimmering of the birches and the swaying of the bamboo,” he says. “There’s a world outside that depends on you developing things that matter.”